Hong Kong Med J 2016 Jun;22(3):270–8 | Epub 22 Apr 2016
DOI: 10.12809/hkmj154678
© Hong Kong Academy of Medicine. CC BY-NC-ND 4.0
REVIEW ARTICLE
Magnetic resonance imaging of the fetal brain
Lawrence MF Tee, FHKCR, FHKAM (Radiology)1;
Elaine YL Kan, FHKCR, FHKAM (Radiology)1;
Joey CY Cheung, MHlthSc (MRS)1;
WC Leung, MD, FRCOG2
1 Department of Diagnostic and Interventional Radiology, Kwong Wah Hospital, Yaumatei, Hong Kong
2 Department of Obstetrics and Gynaecology, Kwong Wah Hospital, Yaumatei, Hong Kong
Corresponding author: Dr Lawrence MF Tee (lmftee@gmail.com)

Abstract
Introduction: This review covers the recent
literature on fetal brain magnetic resonance imaging,
with emphasis on techniques, advances, common
indications, and safety.
Methods: We conducted a search of MEDLINE
for articles published after 2010. The search terms
used were “(fetal OR foetal OR fetus OR foetus)
AND (MR OR MRI OR [magnetic resonance]) AND
(brain OR cerebral)”. Consensus statements from
major authorities were also included. As a result, 44
relevant articles were included and formed the basis
of this review.
Results: One major challenge is fetal motion
that is largely overcome by ultra-fast sequences.
Currently, single-shot fast spin-echo T2-weighted
imaging remains the mainstay for motion resistance
and anatomical delineation. Recently, a snap-shot
inversion recovery sequence has enabled robust T1-weighted images to be obtained, which is previously
a challenge for standard gradient-echo acquisitions.
Fetal diffusion-weighted imaging, diffusion tensor
imaging, and magnetic resonance spectroscopy are
also being developed. With multiplanar capabilities,
superior contrast resolution and field of view,
magnetic resonance imaging does not have the
limitations of sonography, and can provide additional
important information. Common indications include
ventriculomegaly, callosum and posterior fossa
abnormalities, and twin complications. There are
safety concerns about magnetic resonance–induced
heating and acoustic damage but current literature
showed no conclusive evidence of deleterious
fetal effects. The American College of Radiology
guideline states that pregnant patients can be accepted
to undergo magnetic resonance imaging at any stage
of pregnancy if risk-benefit ratio to patients warrants
that the study be performed.
Conclusions: Magnetic resonance imaging of
the fetal brain is a safe and powerful adjunct
to sonography in prenatal diagnosis. It can
provide additional information that aids clinical
management, prognostication, and counselling.
Introduction
Fetal magnetic resonance (MR) imaging has been
an invaluable adjunct to sonography in evaluation
of the fetal brain since its introduction in the 1980s.
In recent years, there has been an exponential
growth in its clinical use, facilitated by technological
advancements such as ultra-fast imaging sequences,
diffusion-weighted imaging (DWI), and parallel
imaging techniques. To date, sonography remains
the mainstay modality in prenatal evaluation,
owing to its low cost, abundant availability, and
well-established literature support. Nonetheless,
MR imaging has been shown to provide useful
complementary information to sonography,1 2
and has a number of advantages over sonography,
including superior contrast resolution, increased
field of view (FOV), and the ability to image
unhampered by an ossified calvarium, large maternal
body habitus, or oligohydramnios.3 4 5 Currently, fetal MR imaging is most commonly used to confirm or
characterise an abnormality that is suspected on
sonography, or to screen fetuses at increased risk of
neurodevelopmental disabilities.
In this article, we review the recent literature
and developments in MR imaging of the fetal brain,
with an emphasis on the safety, imaging techniques
and protocols, and common clinical indications.
Methods
We performed a MEDLINE search for all the relevant
scientific articles published in 2010 or later, using the
keywords “(fetal OR foetal OR fetus OR foetus) AND
(MR OR MRI OR [magnetic resonance]) AND (brain
OR cerebral)”. All papers published in English and on
human subjects were included. This yielded a total
of 331 articles. These were then evaluated for their
content and relevance to this review article, with case
reports being excluded. Ultimately, 40 articles were
deemed relevant and used as the literature basis of
this review. In addition, four consensus statements
and clinical guidelines from major authorities,
including the American College of Radiology (ACR),
the International Commission on Non-Ionizing
Radiation Protection (ICNIRP), the Health and
Safety Executive (HSE), and the American Academy
of Pediatrics, were included. Relevant or important
citations in the articles were also reviewed. With
reference to the data from these articles, we describe
the imaging techniques and recent developments,
common clinical indications, and safety issues of MR
imaging of the fetal brain.
Safety
Fetal imaging is a relatively new MR application.
While it is generally believed to be a safe and noninvasive
procedure,6 safety concerns have been
raised regarding the possible or theoretical adverse
effects related to the radiofrequency (RF) field and
acoustic exposure.7 A number of animal studies have
been performed to assess the safety of MR imaging
in pregnant animals and animal fetuses, but there is
a lack of consensus regarding the actual risks, if any.7
In addition, these studies were performed under
various conditions and MR protocols, making it
difficult to directly extrapolate the results to human
fetal MR examination. Overall, most studies have
shown no deleterious effects of MR imaging on the
developing fetus.4 7 8 9
Radiofrequency-induced hyperthermia
Radiofrequency-induced hyperthermia is a potential
hazard in MR imaging. During an MR examination,
RF energy is converted to heat via Faraday’s law and
is normally dissipated by blood flow. The amount of
heat generated is a function of magnetic field strength,
RF field, body size, tissue resistance, and scan time.
Generally, pregnant women have a similar ability to
maintain heat balance to non-pregnant women in a
warm environment. Since the fetus does not possess
the effective heat dissipation mechanisms of an adult,
fetal temperature is dissipated via amniotic fluid
and umbilical blood flow to the mother, and is thus
coupled to maternal temperature, which is usually
0.5°C higher. Therefore, the fetus is considered
to be more sensitive to hyperthermia. The 2004
ICNIRP report concludes that “Excessive heating is
a potential teratogen; because of uncertainties in the
RF dosimetry during pregnancy, it is recommended
that exposure duration should be reduced to the
minimum and that only the normal operation level
is used”, and that “It seems reasonable to assume that
adverse developmental effects will be avoided with a
margin of safety if the body temperature of pregnant
women does not rise by more than 0.5°C and the
temperature of the fetus is less than 38°C”.10
Acoustic exposure
A characteristic of the switching gradient fields is the
production of acoustic noise. When the alternating
low-frequency currents flow through the gradient
coils, which are immersed in the high static magnetic
field B0, forces are exerted on the gradient coils that
move like a loudspeaker coil and generate sound
waves. Exposure to excessive loud noises can result
in a reduced sensitivity of the hair cells in the organ of
Corti and cause a temporary shift in the threshold of
hearing. The impact depends on the sound pressure
level (measured in A-weighted decibel, dB[A]) and
duration of exposure. A sufficient injurious acoustic
exposure can result in a permanent hearing loss; 85
dB(A) is the threshold for permanent hearing loss
following long-term exposure. Thus, the ICNIRP
recommends that hearing protection should be
provided to patients when sound levels exceed
80 dB(A).10 It is unclear how these guidelines can
be applied to the fetus, in whom the cochlea is
developing, and the external auditory canal and
middle ear cavity are fluid-filled instead of air-filled.
Therefore, concerns have been raised regarding
possible effects of acoustic exposure during fetal
MR on the developing auditory system of the fetus,
especially in echo-planar imaging that is the
loudest sequence in current clinical use.
In the literature reviews published by HSE and
American Academy of Pediatrics, most published
studies were limited by their methodology and
study design, and no conclusive evidence of acoustic
damage was shown.11 12 A 3-year follow-up study of
children who underwent fetal MR imaging showed
no adverse long-term effect on hearing, although
this was limited by the relatively small sample size.7
Compared with adult patients, the exact effect of
acoustic noise on the fetus is difficult to ascertain,
because there are a number of variables that could
alter the effect, for instance, maternal body size,
volume of amniotic fluid, MR sequences used, and
duration of scanning. In a study by Glover et al,13
the authors aimed to simulate the in-utero acoustic
environment by inserting a microphone into the
fluid-filled stomach of a volunteer, and found a >30
dB attenuation in sound intensity. This provides some
reassurance that a level close to the instantaneous
damage threshold (120 dB[A]) could be reduced
to an acceptable level (<90 dB[A]). Overall, the
data from the current literature provide reassuring
clinical and experimental evidence that suggests no
significant risk of acoustic injury to the fetus during
prenatal MR imaging.
Gadolinium contrast medium
Gadolinium is a pregnancy class C drug, and
currently there are no documented indications for
use of gadolinium contrast in fetal MR imaging.9
Gadolinium can pass through the placental barrier
and enter the fetal circulation, with unknown and
potentially harmful effects on the fetus. In animal
studies, large doses of MR imaging gadolinium-based
contrast agents have been shown to be associated
with intrauterine death and congenital anomalies.7
The 2013 ACR guidance document on MR safe
practices states that “MR contrast agents should not
be routinely provided to pregnant patients”.14
Summary
The 2013 ACR guidance document on MR safe
practices states that “Pregnant patients can be
accepted to undergo MR scans at any stage of
pregnancy if, in the determination of a level 2 MR
personnel-designated attending radiologist, the
risk-benefit ratio to the patient warrants that the
study be performed”.14 While present data have not
conclusively documented any deleterious effects of
MR imaging exposure on the developing fetus, as a
precaution, it is generally recommended to wait until
the second trimester onwards before performing
fetal MR imaging. This has the additional benefit of
minimising the technical challenges due to the small
size and excessive motion of younger fetuses.
Techniques and protocols
Coil selection
Fetal MR imaging is typically performed on a 1.5-tesla
magnet. Currently, either multi-channel phased-array
coils or cardiac surface coils are employed for
fetal brain MR, with the coils placed directly over
the fetal head.6 These coils generally lack mechanical
design and flexibility, as maternal size is highly
variable and is also dependent on the gestational
age of the fetus. With the advent of parallel imaging
techniques, the numbers of elements in phased-array
coils have continuously increased to achieve a
higher signal-to-noise ratio (SNR) and shorter scan
times. More recently, the invention of the digital coil
platform combined with multiple coil elements has
further enhanced SNR beyond the capabilities of the
adult eight-channel torso coil routinely used in fetal
imaging.
Patient positioning
Patients are placed in a semi-decubitus position,
rotating about 45° to the left side to avoid compression
of the inferior vena cava and supported with foam
pads to maximise comfort so as to minimise maternal
and fetal movement. No maternal or fetal sedation is
required.
Scout views
Scout images are obtained to localise the fetus and
also serve as a general survey of the feto-placental
unit. An initial localiser is obtained in three
orthogonal planes with respect to the mother, using
6- to 8-mm thick slices with a 1- to 2-mm gap and
a large FOV. The localiser is used to visualise the
position of the fetus and determine fetal sidedness,
as well as to ensure that the coil is centred over
the fetal brain. At our centre, we use a gradient-echo
scout localiser with a large FOV of 450 mm to
cover the abdomen and pelvis of the mother, with a
resolution of 1.76 x 1.76 x 10 mm3, and 20 slices in
three orthogonal planes. A repeat localiser is then
placed in the three orthogonal planes of the fetal
brain. During the examination, each sequence also
serves as a scout for the next. Due to fetal movement
throughout the scanning process, repeating of scout
localisers and repositioning of the coil are sometimes
necessary. Therefore efforts should be made to keep
the scanning time short.
Sequences
High image quality and resolution are crucial to
accurate diagnosis; at the same time, a relatively
short scan time is required to minimise the effects
of fetal movement. Therefore, the choice of technical
parameters should strike a balance between image
quality and scan time (Table).
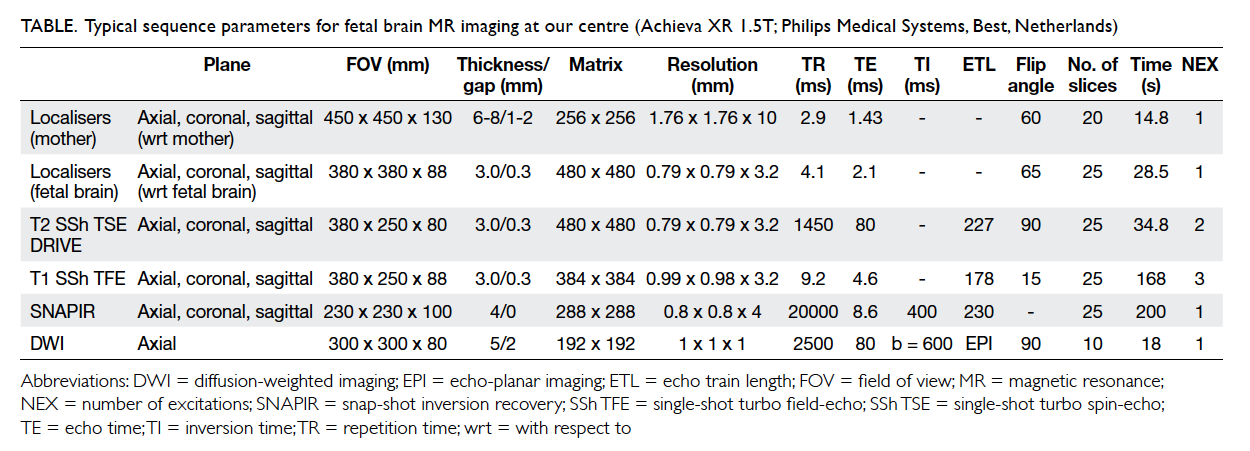
Table. Typical sequence parameters for fetal brain MR imaging at our centre (Achieva XR 1.5T; Philips Medical Systems, Best, Netherlands)
Single-shot fast spin-echo (SSFSE) T2-weighted imaging is regarded as the mainstay of
fetal MRI.3 5 6 8 9 15 16 17 18 19 It provides excellent delineation
of cerebral anatomy and requires a total acquisition
time of <1 second per image. On our 1.5T MR
scanner system (Achieva XR 1.5T; Philips Medical
Systems, Best, Netherlands), SSFSE T2-weighted
sequences in the axial, coronal, and sagittal plane
of the fetal brain are acquired, with 25 slices in 35
seconds, resolution at 0.79 x 0.79 x 3.2 mm, 0.3
mm gap, long echo train length (ETL) of 227 and
relatively short repetition time (TR) of 1450 ms, and
use of the driven equilibrium pulse to enhance T2
contrast with a shorter TR.
Single-shot multiplanar T1-weighted turbo field-echo sequences are acquired to detect
haemorrhage, fat, or calcification.3 5 6 8 9 Snap-shot
inversion recovery, a dedicated optimised inversion-recovery-prepared SSFSE T1-weighted sequence,
offers detailed delineation of normal fetal brain
anatomy and myelination near term.20 At our centre,
we use an inversion time of about 400 ms, resolution
at 0.8 x 0.8 x 4 mm, 25 slices in 3 minutes 20 seconds,
and single-shot mode with ETL of 230.
Diffusion-weighted imaging provides
quantitative information about water motion and
tissue microstructure, and can be used to identify
focal areas of injury and delineate subtle anatomical
structures and maturational changes.3 8 15 21 22 Other
advanced techniques are also being developed and
may provide functional and physiological information,
including fetal MR spectroscopy, diffusion tensor
imaging, and functional imaging,7 8 23 24 25 26 27 28 29 30 although their
development is still at an early stage.
Indications
Fetal MR imaging is often performed to further
evaluate a suspected abnormality detected on
sonography. By providing additional information on
the suspected abnormality and detecting associated
cerebral abnormalities that are otherwise occult on
sonography, fetal MR imaging can guide antenatal
and perinatal management, as well as assist in the
counselling of current and future pregnancies.5 31 32 33 34
It has also been shown to demonstrate a high
diagnostic accuracy when compared with repeat
or postnatal MR imaging.35 36 The most common
clinical indications for fetal MR imaging will be
discussed below.
Ventriculomegaly
Ventriculomegaly is one of the most common
clinical indications for fetal MR imaging, mainly
to detect other associated abnormalities that are
occult on sonography. Ventriculomegaly is defined
as atrial width of >10 mm on sonography, measured
in the axial plane, at the level of the frontal horns
and cavum septi pellucidi, at the level of the glomus
of the choroid plexus, and perpendicular to the long
axis of the lateral ventricle.3 5 6
Ventriculomegaly is a relatively common
abnormality detected on prenatal sonography. It is a
heterogeneous disease with various aetiologies that
can be classified into developmental, destructive, and
obstructive pathologies. An important prognostic
factor is whether the ventriculomegaly is isolated,
or with additional associated abnormalities.26
Studies have shown that up to 80% of fetuses
with ventriculomegaly have other associated
central nervous system (CNS) abnormalities, and
is associated with poor postnatal neurological
and developmental outcomes3 37 (Fig 1). These
include agenesis of the corpus callosum, cortical
malformation, periventricular nodular heterotopia,
and destructive processes such as periventricular
leukomalacia, porencephaly, and intra-ventricular
and subependymal haemorrhage.3 6 37 On the other
hand, the majority of fetuses with isolated mild
ventriculomegaly show normal neurodevelopmental
outcomes. Fetal MR imaging has been shown to
have a high sensitivity and specificity for these brain
abnormalities18 that may be occult and unidentified
on sonography.25 34 38 39 40 These data highlight the
role of fetal MR imaging in the prognostication of
ventriculomegaly, and have significant implications
on parental counselling and perinatal management.
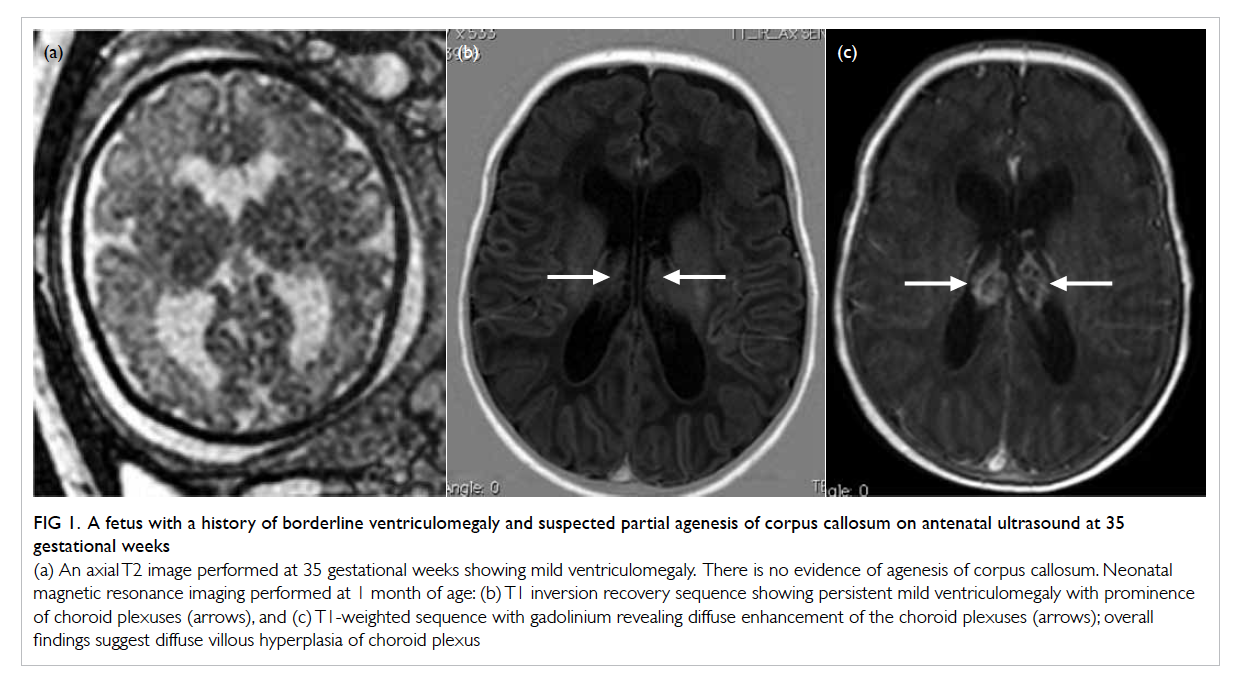
Figure 1. A fetus with a history of borderline ventriculomegaly and suspected partial agenesis of corpus callosum on antenatal ultrasound at 35 gestational weeks
(a) An axial T2 image performed at 35 gestational weeks showing mild ventriculomegaly. There is no evidence of agenesis of corpus callosum. Neonatal magnetic resonance imaging performed at 1 month of age: (b) T1 inversion recovery sequence showing persistent mild ventriculomegaly with prominence of choroid plexuses (arrows), and (c) T1-weighted sequence with gadolinium revealing diffuse enhancement of the choroid plexuses (arrows); overall findings suggest diffuse villous hyperplasia of choroid plexus
Corpus callosum abnormalities
The corpus callosum is the main commissural
pathway in the brain and comprises the rostrum,
genu, body, and splenium. Beginning at 8 weeks of
gestation, it develops from the lamina of His, with
an apparent anteroposterior progression, starting
from the genu, progressing posteriorly to the body
and splenium, followed by the rostrum. By 15 to 20
weeks, the corpus callosum has assumed its final
shape with fusion of all of its parts.6 Thus caution
should be exercised when evaluating the corpus
callosum before 20 weeks.
Abnormalities of the corpus callosum include
agenesis, hypogenesis, dysgenesis, hypoplasia,
and destruction. The precise incidence of corpus
callosum abnormalities is difficult to ascertain
because of selection bias in reported series. In a
large population-based study looking at data from
the California Birth Defect Monitoring Program,41
the authors identified 630 (0.019%) cases of agenesis
or hypoplasia of the corpus callosum in 3.4 million
live births. While agenesis of the corpus callosum
can sometimes be seen in asymptomatic individuals,
most patients exhibit variable neurological
symptoms, including developmental delay,
cognitive impairment, and epilepsy. There is also
a high association with other CNS abnormalities
such as sulcation abnormalities, Dandy-Walker
malformation, Chiari II malformation, and grey
matter heterotopia.3 6 9
On sonography, the corpus callosum is best
visualised and evaluated on the mid-sagittal image,
but obtaining an optimal mid-sagittal view can be
challenging, especially at an advanced gestational
age. We often rely on indirect signs including
absence of cavum septum pellucidum, colpocephaly,
high-riding third ventricle, and radiating gyri.3 6
Fetal MR imaging overcomes these challenges
with its multiplanar capabilities and is able to
demonstrate the corpus callosum in its entire
length on the mid-sagittal image as a curvilinear
C-shaped T2 hypointense structure at the superior
margin of the cavum septum pellucidum and lateral
ventricles.3 The indirect signs of callosal agenesis,
similar to those on sonography, can also be depicted
on the axial and coronal images3 6 (Fig 2). Studies
have shown a higher sensitivity and specificity with
fetal MR imaging compared with sonography.38 42
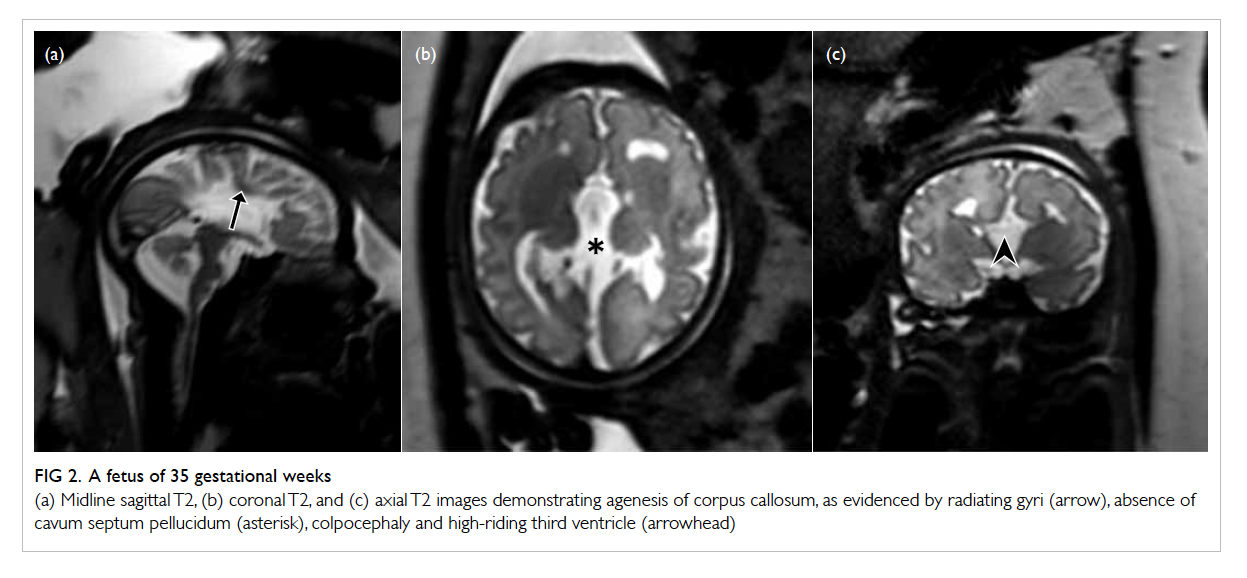
Figure 2. A fetus of 35 gestational weeks
(a) Midline sagittal T2, (b) coronal T2, and (c) axial T2 images demonstrating agenesis of corpus callosum, as evidenced by radiating gyri (arrow), absence of cavum septum pellucidum (asterisk), colpocephaly and high-riding third ventricle (arrowhead)
The prognosis of corpus callosum
abnormalities is highly variable, depending not
on the callosal abnormality itself, but largely on
the associated abnormalities in the CNS and other
systems, and have been found to be as common as
85% on autopsy.5 Fetal MR imaging has been shown
to demonstrate additional but sonographically
occult anomalies in up to 93% of cases of callosal
abnormalities.5 9 Accurate detection of associated
abnormalities has an important implication on the
prognostication of the current pregnancy and the
recurrence risk in future pregnancies.3 5
Complications of monochorionic twin pregnancies
While fetal MR imaging is commonly used for
further evaluation of suspected sonographic
abnormalities, there has been an increasing clinical
use in the screening of high-risk cases.3 31 43 Fetal MR imaging is particularly useful in monochorionic twin
pregnancies complicated by twin-twin transfusion
syndrome or co-twin fetal demise where sonography
of the brain is unrevealing.43 44
In twin-twin transfusion syndrome, there
is imbalanced blood flow from the smaller donor
twin to the larger recipient twin via abnormal
intertwin vascular connections in the shared
common monochorionic placenta. The donor
twin develops oliguria and oligohydramnios
from volume depletion, while the recipient twin
develops polyuria and polyhydramnios from
volume overload. High morbidity and mortality
are observed, with both donor and recipient
twins at a higher risk of cerebral ischaemia and
haemorrhage, and neurodevelopmental and
sonographic abnormalities.3 44 Although imaging the
oligohydramniotic twin is usually straightforward,
imaging the polyhydramniotic twin can be difficult
owing to excessive fetal motion.
In co-twin fetal demise, an increased risk of
neurological impairment is seen in the surviving
co-twin (Fig 3). The likely mechanisms of cerebral
injury are believed to involve an acute haemodynamic
disturbance due to exsanguination of the surviving
co-twin into the dead fetus just before or at the time
of fetal demise, as well as thromboembolic events at
the time of demise.3 43
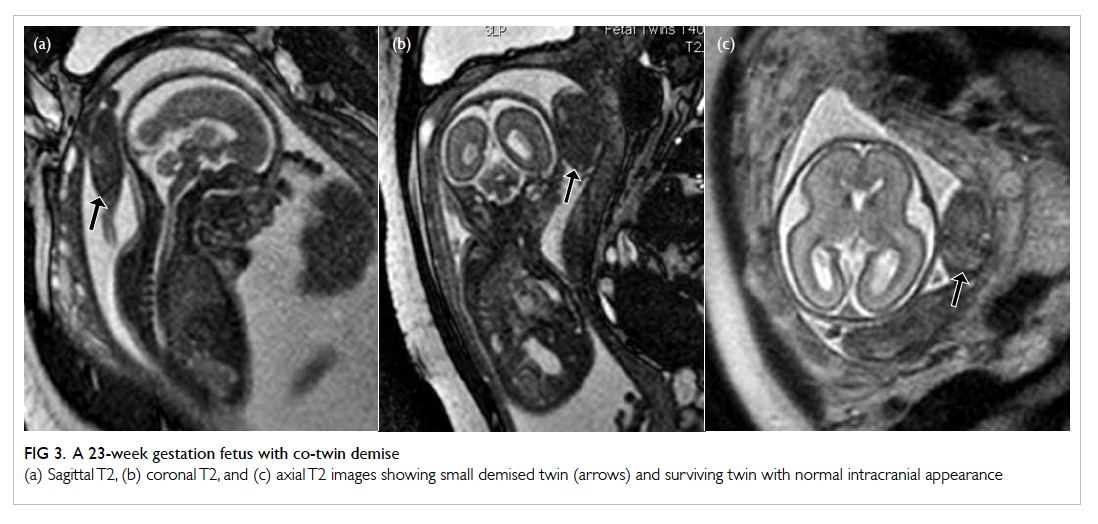
Figure 3. A 23-week gestation fetus with co-twin demise
(a) Sagittal T2, (b) coronal T2, and (c) axial T2 images showing small demised twin (arrows) and surviving twin with normal intracranial appearance
Because of the high morbidity in these twin
pregnancy complications, fetal MR imaging can be
employed to look for cerebral injuries even when
sonography appears normal, such as periventricular
leukomalacia, encephalomalacia, germinal matrix
haemorrhage, intra-ventricular haemorrhage,
intraparenchymal haemorrhage, and cortical
malformations. It has been found that one third
of the surviving twins of co-twin fetal demise had
abnormal cerebral findings on fetal MR imaging;
most of which were occult sonographically.41 In
addition, early manifestations of cerebral ischaemia
were better diagnosed with MR imaging than
sonography, especially DWI.3 43
Posterior cranial fossa abnormalities
Fetal MR imaging is useful in evaluating the posterior
cranial fossa, utilising its ability to directly visualise
the cerebellar hemisphere, vermis, and brainstem in
three orthogonal planes, providing global assessment
of the posterior fossa structures with morphologic
and biometric analysis. It is also used to evaluate
for supratentorial abnormalities that are commonly
associated with various posterior fossa diseases, and
can aid diagnosis and prognostication. Posterior
fossa abnormalities that can be evaluated by fetal
MR imaging include Dandy-Walker spectrum,
cerebellar hypoplasia, cerebellar dysplasia, cerebellar
haemorrhage, and Chiari malformation.5 6 9 45
Dandy-Walker malformation is characterised
by agenesis or hypoplasia of the cerebellar vermis,
in association with an enlarged posterior fossa,
torcular-lambdoid inversion, and cystic dilatation of
the fourth ventricle.5 6 9 While severe cases of Dandy-Walker malformation can be readily identified
by sonography, distinguishing milder forms of
vermian hypoplasia from a mega cisterna magna
or an arachnoid cyst can be challenging (Figs 4 and
5). This is even more difficult in the third trimester
where ossification of the skull can limit sonographic
assessment of the posterior fossa structures. With
its multiplanar capabilities, fetal MR imaging can
better evaluate the morphology of the vermis,
as well as the anatomical relationship between a
retrocerebellar cyst and the fourth ventricle, which
can help differentiate a Dandy-Walker variant from
other entities such as a mega cisterna magna.5 25 In
addition, fetal MR imaging is able to evaluate the
supratentorial structures, because the Dandy-Walker
spectrum is also associated with supratentorial
abnormalities such as agenesis of corpus callosum,
polymicrogyria, neuronal heterotopia, and occipital
encephalocele,5 and is associated with a poorer
clinical outcome. On the other hand, radiologists
should also be aware of the limitations of fetal
MR imaging. At younger gestational age (such as
<20 weeks), fetal MR imaging may have a reduced
specificity, particularly in the diagnosis of isolated
inferior vermian hypoplasia.5 9 This may be related
to small size, fetal motion, volume averaging, and
difficulty in obtaining a true mid-sagittal image.
Follow-up MR imaging, either at a later gestational
age or postnatally, is recommended in such cases.46
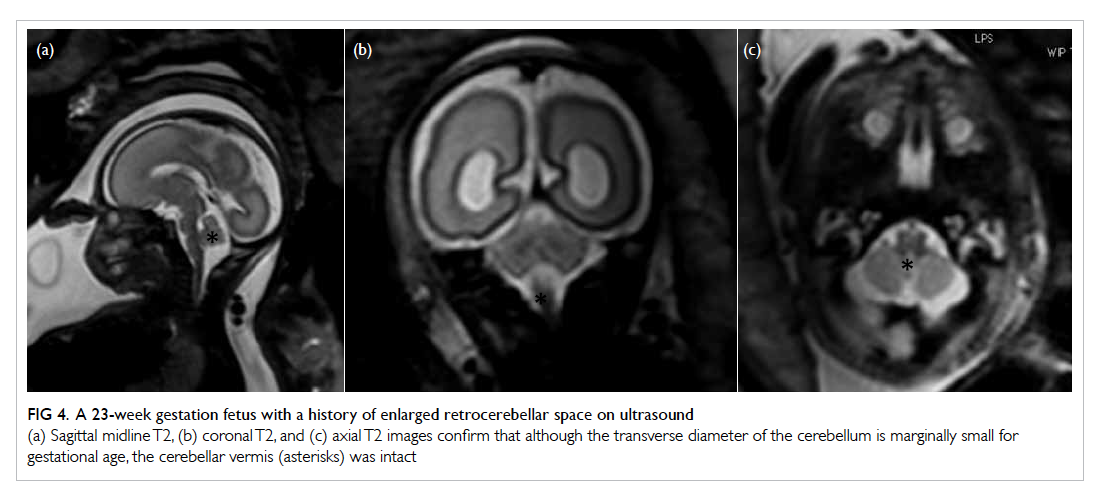
Figure 4. A 23-week gestation fetus with a history of enlarged retrocerebellar space on ultrasound
(a) Sagittal midline T2, (b) coronal T2, and (c) axial T2 images confirm that although the transverse diameter of the cerebellum is marginally small for gestational age, the cerebellar vermis (asterisks) was intact
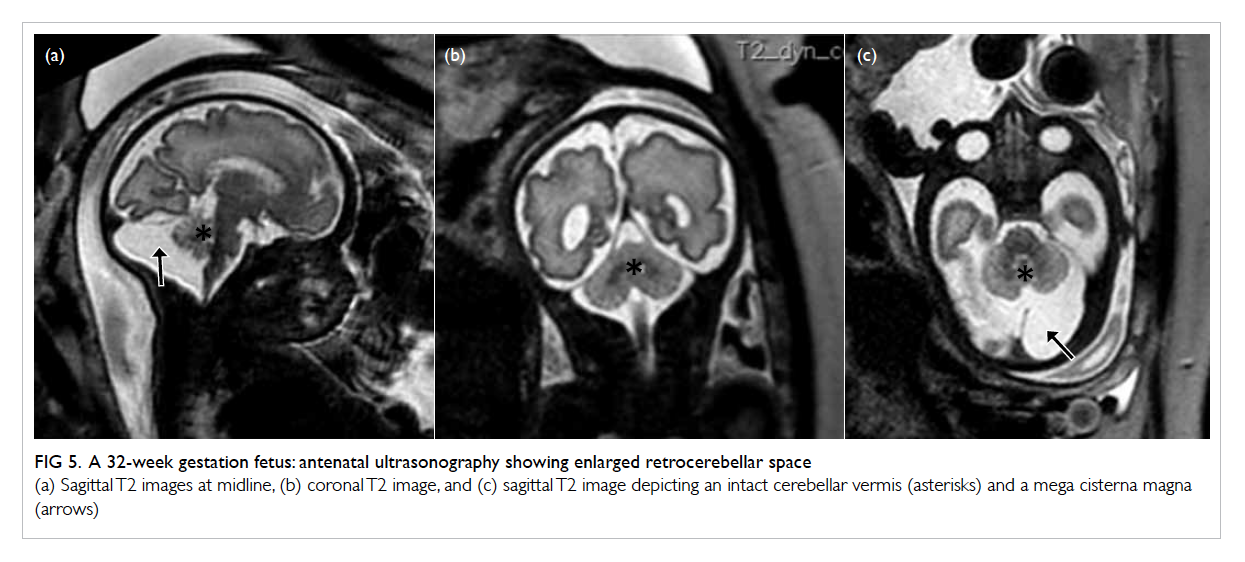
Figure 5. A 32-week gestation fetus: antenatal ultrasonography showing enlarged retrocerebellar space
(a) Sagittal T2 images at midline, (b) coronal T2 image, and (c) sagittal T2 image depicting an intact cerebellar vermis (asterisk) and a mega cisterna magna (arrow)
The cerebellar hemispheres can be evaluated
on fetal MR imaging by assessing their size and
morphology in multiple planes, in which normative
data have been published for different gestational
ages.3 Furthermore, DWI can provide quantitative
information on the developing cerebellum that
normally demonstrates a progressive decline in
diffusivity and apparent diffusion coefficient values
with increasing gestational age.
Fetal MR imaging is also helpful in evaluating
echogenic posterior fossa masses. Haemorrhage
is typically hyperintense on T1-weighted images,
hypointense on T2-weighted images, with
susceptibility artefact on gradient echo T2* images,
although the signal intensity can vary depending
on the age of the haemorrhage. In addition to
confirming the diagnosis, fetal MR imaging can also
better delineate the location of the haemorrhage,
whether it is intra-axial or extra-axial which have
different pathophysiology. The underlying causes of
the cerebellar haemorrhage can be evaluated on MR
imaging, such as germinal matrix haemorrhages,
vascular malformations, and congenital infections.38
Conclusions
Magnetic resonance imaging is a safe and powerful
adjunct to sonography in prenatal evaluation of
the fetal brain. Facilitated by recent technological
advancements, fetal MR imaging is being
increasingly used in the clinical evaluation of cerebral
abnormalities and screening of high-risk fetuses. It
can provide additional useful information that can
alter clinical management and aid in prognostication
and counselling. Radiologists and clinicians
involved in prenatal imaging and management
should be aware of the application and limitations
of the modalities available in fetal imaging, so as to
optimise the multidisciplinary care for our patients.
Acknowledgement
The authors thank Professor Mary Rutherford,
Centre for the Developing Brain, Perinatal Imaging &
Health Imaging Sciences & Biomedical Engineering
Division, King’s College London, St Thomas’
Hospital, London, United Kingdom.
References
1. Blondiaux E, Sileo C, Nahama-Allouche C, et al.
Periventricular nodular heterotopia on prenatal ultrasound
and magnetic resonance imaging. Ultrasound Obstet
Gynecol 2013;42:149-55. Crossref
2. Blondiaux E, Garel C. Fetal cerebral imaging—ultrasound
vs. MRI: an update. Acta Radiol 2013;54:1046-54. Crossref
3. Glenn OA. MR imaging of the fetal brain. Pediatr Radiol
2010;40:68-81. Crossref
4. Mirsky DM, Shekdar KV, Bilaniuk LT. Fetal MRI: head and
neck. Magn Reson Imaging Clin N Am 2012;20:605-18. Crossref
5. Kline-Fath BM, Calvo-Garcia MA. Prenatal imaging of
congenital malformations of the brain. Semin Ultrasound
CT MR 2011;32:167-88. Crossref
6. Saleem SN. Fetal magnetic resonance imaging (MRI): a
tool for a better understanding of normal and abnormal
brain development. J Child Neurol 2013;28:890-908. Crossref
7. Bulas D, Egloff A. Benefits and risks of MRI in pregnancy.
Semin Perinatol 2013;37:301-4. Crossref
8. Mailath-Pokorny M, Kasprian G, Mitter C, Schöpf V,
Nemec U, Prayer D. Magnetic resonance methods in fetal
neurology. Semin Fetal Neonatal Med 2012;17:278-84. Crossref
9. O’Connor SC, Rooks VJ, Smith AB. Magnetic resonance
imaging of the fetal central nervous system, head, neck,
and chest. Semin Ultrasound CT MR 2012;33:86-101. Crossref
10. International Commission on Non-Ionizing Radiation
Protection. Medical magnetic resonance (MR) procedures:
protection of patients. Health Phys 2004;87:197-216. Crossref
11. Health and Safety Executive. Non-auditory effects of noise
at work: a critical review of the literature. Sudbury: HSE
Books; 1999.
12. Noise: a hazard for the fetus and newborn. American
Academy of Pediatrics. Committee on Environmental
Health. Pediatrics 1997;100:724-7. Crossref
13. Glover P, Hykin J, Gowland P, Wright J, Johnson I, Mansfield
P. An assessment of the intrauterine sound intensity level
during obstetric echo-planar magnetic resonance imaging.
Br J Radiol 1995;68:1090-4. Crossref
14. Expert Panel on MR Safety, Kanal E, Barkovich AJ, Bell C,
et al. ACR guidance document on MR safe practices: 2013.
J Magn Reson Imaging 2013;37:501-30. Crossref
15. Williams F, Griffiths PD. The diagnosis of
hemimegalencephaly using in utero MRI. Clin Radiol
2014;69:e291-7. Crossref
16. Griffiths PD, Jarvis D, McQuillan H, Williams F, Paley
M, Armitage P. MRI of the foetal brain using a rapid 3D
steady-state sequence. Br J Radiol 2013;86:20130168. Crossref
17. Malamateniou C, Malik SJ, Counsell SJ, et al. Motion-compensation
techniques in neonatal and fetal MR imaging. AJNR Am J Neuroradiol 2013;34:1124-36. Crossref
18. Glenn OA, Cuneo AA, Barkovich AJ, Hashemi Z, Bartha AI,
Xu D. Malformations of cortical development: diagnostic
accuracy of fetal MR imaging. Radiology 2012;263:843-55. Crossref
19. Shekdar K, Feygin T. Fetal neuroimaging. Neuroimaging
Clin N Am 2011;21:677-703, ix. Crossref
20. Clouchoux C, Limperopoulos C. Novel applications of
quantitative MRI for the fetal brain. Pediatr Radiol 2012;42
Suppl 1:S24-32. Crossref
21. Mignone Philpott C, Shannon P, Chitayat D, Ryan G,
Raybaud CA, Blaser SI. Diffusion-weighted imaging of the
cerebellum in the fetus with Chiari II malformation. AJNR
Am J Neuroradiol 2013;34:1656-60. Crossref
22. Kasprian G, Del Río M, Prayer D. Fetal diffusion
imaging: pearls and solutions. Top Magn Reson Imaging
2010;21:387-94. Crossref
23. Story L, Damodaram MS, Supramaniam V, et al. Myo-inositol
metabolism in appropriately grown and growth-restricted
fetuses: a proton magnetic resonance spectroscopy study.
Eur J Obstet Gynecol Reprod Biol 2013;170:77-81. Crossref
24. Vasung L, Fischi-Gomez E, Huppi PS. Multimodality
evaluation of the pediatric brain: DTI and its competitors.
Pediatr Radiol 2013;43:60-8. Crossref
25. Girard NJ. Magnetic resonance imaging of fetal
developmental anomalies. Top Magn Reson Imaging
2011;22:11-23. Crossref
26. Huisman TA. Fetal magnetic resonance imaging of the
brain: is ventriculomegaly the tip of the syndromal iceberg?
Semin Ultrasound CT MR 2011;32:491-509. Crossref
27. Story L, Damodaram MS, Allsop JM, et al. Brain metabolism
in fetal intrauterine growth restriction: a proton magnetic
resonance spectroscopy study. Am J Obstet Gynecol
2011;205:483.e1-8. Crossref
28. Meoded A, Poretti A, Tekes A, Flammang A, Pryde S,
Huisman TA. Prenatal MR diffusion tractography in a fetus
with complete corpus callosum agenesis. Neuropediatrics
2011;42:122-3. Crossref
29. Schöpf V, Kasprian G, Prayer D. Functional imaging in the
fetus. Top Magn Reson Imaging 2011;22:113-8. Crossref
30. Story L, Damodaram MS, Allsop JM, et al. Proton magnetic
resonance spectroscopy in the fetus. Eur J Obstet Gynecol
Reprod Biol 2011;158:3-8. Crossref
31. Griffiths PD, Porteous M, Mason G, et al. The use of in
utero MRI to supplement ultrasound in the foetus at high
risk of developmental brain or spine abnormality. Br J
Radiol 2012;85:e1038-45. Crossref
32. Doneda C, Parazzini C, Righini A, et al. Early cerebral
lesions in cytomegalovirus infection: prenatal MR imaging.
Radiology 2010;255:613-21. Crossref
33. Lipitz S, Hoffmann C, Feldman B, Tepperberg-Dikawa
M, Schiff E, Weisz B. Value of prenatal ultrasound and
magnetic resonance imaging in assessment of congenital
primary cytomegalovirus infection. Ultrasound Obstet
Gynecol 2010;36:709-17. Crossref
34. Griffiths PD, Reeves MJ, Morris JE, et al. A prospective
study of fetuses with isolated ventriculomegaly investigated
by antenatal sonography and in utero MR imaging. AJNR
Am J Neuroradiol 2010;31:106-11. Crossref
35. Dhouib A, Blondiaux E, Moutard ML, et al. Correlation
between pre- and postnatal cerebral magnetic resonance
imaging. Ultrasound Obstet Gynecol 2011;38:170-8. Crossref
36. Griffiths PD, Morris JE, Mason G, et al. Fetuses with
ventriculomegaly diagnosed in the second trimester of
pregnancy by in utero MR imaging: what happens in the
third trimester? AJNR Am J Neuroradiol 2011;32:474-80. Crossref
37. Mehrabi S, Adami A, Ventriglia A, Zantedeschi L, Franchi
M, Manfredi R. Evolution of ventriculomegaly: comparison
between foetal MR imaging and postnatal diagnostic
imaging. Radiol Med 2013;118:1199-211. Crossref
38. Manganaro L, Bernardo S, La Barbera L, et al. Role of foetal
MRI in the evaluation of ischaemic-haemorrhagic lesions
of the foetal brain. J Perinat Med 2012;40:419-26. Crossref
39. Manfredi R, Tognolini A, Bruno C, Raffaelli R, Franchi M,
Pozzi Mucelli R. Agenesis of the corpus callosum in fetuses
with mild ventriculomegaly: role of MR imaging. Radiol
Med 2010;115:301-12. Crossref
40. Yin S, Na Q, Chen J, Li-Ling J, Liu C. Contribution of MRI
to detect further anomalies in fetal ventriculomegaly. Fetal
Diagn Ther 2010;27:20-4. Crossref
41. Glass HC, Shaw GM, Ma C, Sherr EH. Agenesis of the
corpus callosum in California 1983-2003: a population-based
study. Am J Med Genet A 2008;146A:2495-500. Crossref
42. Griffiths PD, Russell SA, Mason G, Morris J, Fanou E,
Reeves MJ. The use of in utero MR imaging to delineate
developmental brain abnormalities in multifetal
pregnancies. AJNR Am J Neuroradiol 2012;33:359-65. Crossref
43. Hoffmann C, Weisz B, Yinon Y, et al. Diffusion MRI findings
in monochorionic twin pregnancies after intrauterine fetal
death. AJNR Am J Neuroradiol 2013;34:212-6. Crossref
44. Tarui T, Khwaja OS, Estroff JA, Robinson JN, Gregas
MC, Grant PE. Altered fetal cerebral and cerebellar
development in twin-twin transfusion syndrome. AJNR
Am J Neuroradiol 2012;33:1121-6. Crossref
45. Righini A, Parazzini C, Doneda C, et al. Fetal MRI features
related to the Chiari malformations. Neurol Sci 2011;32
Suppl 3:S279-81. Crossref
46. Guibaud L, Larroque A, Ville D, et al. Prenatal diagnosis
of ‘isolated’ Dandy-Walker malformation: imaging findings
and prenatal counselling. Prenat Diagn 2012;32:185-93. Crossref